A detailed look at the most probable path forward
Alex Nedelcu and Miguel Castro Gracia, Leonardo Times Editors
Quoting the International Air Transport Association, in 2024, “there was no let-up in the industry’s determination to achieve net zero carbon emissions by 2050” [1]. But beyond the promotional material, is the aviation sector really on the way to decarbonizing in time to avoid the most dangerous climate scenarios? Or is the industry’s continued growth fundamentally incompatible with sustainable transport?
Commercial aviation has increased more than eighty-fold since the 1960s, with a 3% average yearly growth rate. It has already contributed around 3.5% of the total anthropogenic global warming to date and now contributes around 5.9% of ongoing emissions. While global warming is generally considered a problem of carbon dioxide, aviation’s CO2 emissions have been found to only represent a third of its total contribution to global warming, with the non-CO2 contribution flowing down from nitrogen oxide, water vapor, and contrails [2].
The Paris Agreement set a target to keep global warming below 2 degrees Celsius over pre-industrial levels. As such, aviation, like all other emitting sectors of human society, must decarbonize and reduce its impact on the Earth system. In this article, we apply a simple quantitative framework to evaluate the prospects of aviation decarbonizing in what we consider to be the most probable scenario.
Methodology
Studying the commercial aviation sector in detail is an enormous undertaking. Therefore, in order to quickly draw useful conclusions, our objective is to create a simple, intuitive framework for the climate impact of aviation. In transport ecology, the study of transport mode impacts is frequently pursued quantitatively through the Kaya identity, which allows for the impact to be divided into its component quantities [3]:
Impact=DemandVehiclesPassengersEnergy SpentDistance TraveledTotal EmissionsEnergy Spent
Where demand is measured in passengers-kilometer, vehicles/passenger represents the inverse of occupancy in passenger-kilometers per vehicle-kilometers, energy spent/distance traveled represents the inverse of energy efficiency (in km/kWh), and total emissions/energy spent represents the carbon intensity (in CO2-equivalent/kWh). Because of the fundamental differences in demand, occupancy, and technological opportunities between long-haul and short-haul flights, we will investigate each separately, using a loose definition of short-haul flights as those under around 1500-2000 kilometers and long-haul flights as those above 2000-2500 kilometers.
Though we aim to reach quantitative conclusions by separating the component quantities, it is unlikely that complete and certain data is available for all existing (and especially prospective) sections. Many predictions, including future macroeconomic growth and technological development, are bristling with uncertainty. In this context, we realize that such a back-of-the-envelope estimation is inherently subjective and driven by the author’s biases. However, we believe it can still provide a qualitative framework for thinking seriously about the feasibility of decreasing aviation’s environmental impact.
Though scenario analysis could be in some cases exploratory [4], and investigate possible futures (for example, looking at possible pathways toward decarbonization based on how the technological, social, and economic environment would develop), our objective is to take a predictive look at the future. This means accounting for current material conditions in and around the aviation sector to extrapolate the most probable scenario out of the possible pathways forward to the best of our ability. This approach contrasts with a comprehensive paper by Bergero et al. [5], which studies many possible pathways toward decarbonization with a similar framework. With these caveats out of the way, the first (and least optimistic) quantity to be studied is future demand for commercial aviation.
Demand
In the industry, demand is represented in “Revenue Passengers-Kilometer”, meaning the total number of paying passengers multiplied by the kilometers they travel. The 2019 RPK value of 8.4 trillion is typically used as a reference, which is on the way to being met once again in 2024 after the disruption caused by the COVID-19 pandemic. The International Air Transport Association, in its 2024 annual report [1], predicts an average annual growth rate of 3.8% until 2043, while Bain & Company estimates an overall increase in RPK of 36% by 2030 compared to 2019 numbers [6], resulting in a total RPK of 11.4 trillion. Eurocontrol estimates that long-haul flights cover around 40% of the total RPK [7], so a 60-40 division of demand between long-haul and short-haul sounds reasonable, resulting in 3.4 trillion RPK for short-haul and 5 trillion RPK for long-haul flights for 2019. This means that, even though there are fewer long-haul flights, their greater length means that emissions are more or less balanced between the two.
Whether led by general macroeconomic growth, increases in spending power, or higher competition by low-cost carriers, any kind of increase in demand will result in a proportional impact increase. According to our simple framework, IATA’s estimate of 3.8% growth, approximately in agreement with historical figures for growth, would result in an almost tripled demand by 2050. Of course, a constant growth rate is simply an extrapolation of current trends and excludes uncertainties related to the destabilization of the climate system, geopolitical and policy events, or flash technological breakthroughs. However, all scenarios seem to point toward continuous growth. Whether this growth will (or even can) be curtailed is a topic we keep for later.
Occupancy
The vehicle-kilometers per passenger-kilometers value is the inverse of the occupancy, given by the number of passengers per vehicle. To obtain a first-order estimate, we can multiply the average number of passengers by the passenger load factor for the flight. According to ICAO data [8], for short-haul flights, the average aircraft can house 160 passengers (148 for the Boeing 737, 165 for the Airbus A320 etc.), with a passenger load factor of around 0.8. In contrast, long-haul flights can carry around 305 passengers (370 for the Boeing 777, 287 for the Airbus A330 etc.) with a passenger load factor of around 0.82. This means we currently see values of 0.0078 vehicle-kilometers per passenger-kilometers for short-haul flights and 0.004 for long-haul flights. Strictly from the occupancy perspective, a long-haul flight is more sustainable, since it can carry almost double the passengers.
The passenger load factor is the ratio between the revenue passenger kilometers and the available seat kilometers (ASK), with the latter representing the overall supply of seats in commercial aviation. Increasing this ‘efficiency’ beyond 0.8-0.82 would require significantly more advanced passenger traffic forecasting and flight and route planning, which is the subject of much research in the machine learning field [9]. However, the ideal value of 1 remains elusive, as long as there is one unsold seat on a flight.
Energy Efficiency
Zheng and Rutherford find that, since 1970, the fuel burn of aircraft has decreased at a rate of about 1% a year due to the introduction of highly efficient aerodynamic shapes and high-bypass turbofan engines [10]. Their analysis shows that every subsequent aircraft model introduced results in a certain increase in energy efficiency, with the latest being the Airbus A320neo and the Boeing 737 MAX. These incremental improvements lead to the ICAO industry goals being represented by a yearly increase of 2% in energy efficiency [11]. Though Bergero et al. mentions other possible improvements, such as open-rotor power plants, blended wing body aircraft, and other novel architectures resulting in higher possible energy intensity gains [5], we posit that the introduction of these solutions would require serious delays in engineering, certification, and rollout. As such, we believe the ICAO 2% energy efficiency target represents the upper limit of plausibility.
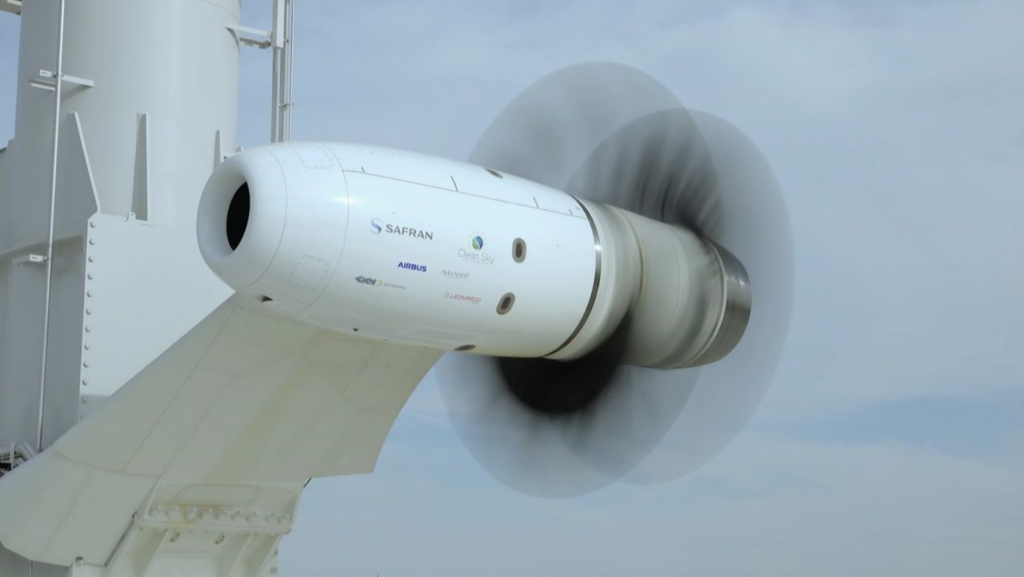
Incremental gains in energy efficiency appear with the rollout of new aircraft models from original equipment manufacturers, resulting in staggered increases as the new models are released and slowly replace the existing aircraft fleet. Achieving a result between the 1% business-as-usual figure and the ICAO 2% figure would decrease from the current energy efficiency of 3.5kWh/km to between 2.7 and 2.1kWh/km by 2050. To represent increased ambition to reduce the energy intensity of aircraft, we believe it probable that a yearly increase of 1.5% efficiency will be met, while not necessarily meeting the ICAO goals – resulting in a final expected value of 2.35kWh/km, or a decrease of about 33% in energy intensity.
These figures do not account for any possible revolutionary improvements, such as the integration of either hydrogen or electricity as energy carriers (discussed in further detail below), which might require the implementation of different, less efficient architectures to account for lower energy density or specialized storage requirements.
Carbon Intensity
Currently, the energy density, vast existing infrastructure, and low cost of kerosene make it an ideal jet fuel. However, regardless of the fuel’s source (fossil, biomass, or synthetic), its combustion inevitably produces CO2 emissions and other particulates that influence global warming. The carbon intensity of jet fuel has remained constant at around 0.265 kilograms of CO2 per kWh [5]. Comparatively, sustainable aviation fuels (SAF), hydrogen, and batteries are touted as lower-carbon alternatives. Sustainable aviation fuels are a topic of great controversy, as the industry, policymakers, and researchers do not agree on their actual decrease in emissions. IATA data shows that only around 0.2% of used fuels are currently biofuels [1], with growth limited by economic conditions, the trade-off between energy crops and food crops, and the inherent limitation of waste-based biofuels. As such, we do not consider that it is possible to provide a reasonable quantitative estimate for the evolution of sustainable aviation fuels, and, together with other non-technological improvements such as offsets and direct air capture, we leave it for further discussion.
Battery-powered aircraft emissions can usually be assumed to be represented by those of the grid that supports them, so a grid with strong support from solar, wind, hydropower, and nuclear electricity generation should theoretically be able to provide sufficient power for running a strong battery-electric aviation subsector. Therefore, for our analysis we will estimate that battery-electric aircraft by 2050 will be carbon-neutral while noting concerns about embodied emissions and the grid energy mix.
However, the viability of battery-powered aircraft is greatly affected by energy density. Battery-powered aircraft suffer from having almost an order of magnitude lower energy density by mass when compared to jet fuel (from 144 kWh/kg to 11 kWh/kg), which makes the concept of long-range battery-powered aircraft impossible with current technologies. This leaves the electric powerplant relegated to a relatively smaller (albeit still important) niche in very short-range flights, with designs currently in commercial production reaching up to 250 km [14].
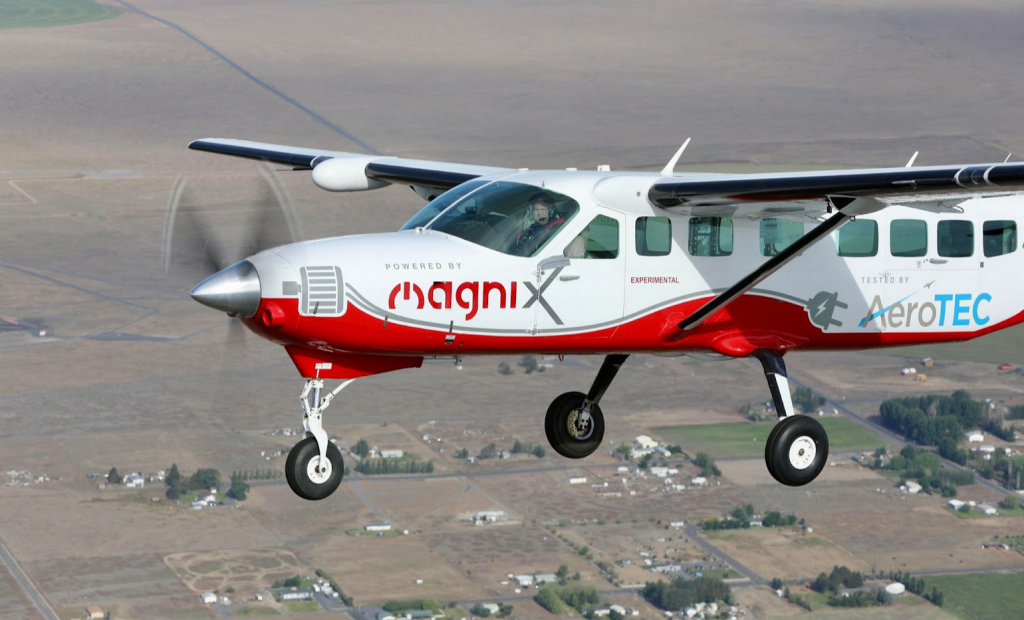
Hydrogen combustion, on the other hand, causes significantly lower emissions than kerosene, and recent research has found that long-haul hydrogen aircraft designs could be more efficient than their kerosene counterparts in terms of energy consumption [12]. However, this does not consider the fuel production itself: the production and liquefaction of hydrogen is currently an energy-intensive industrial process relying on feedstocks of methane. With emissions of 2 kg of CO2 per kilogram of hydrogen, and 33.33kWh per kilogram of hydrogen, the emissions of hydrogen come out to be 0.06 kgCO2/kWh [13] 23% that of kerosene. Additionally, hydrogen aircraft are affected by poor volumetric energy density, resulting in hydrogen aircraft generally having a much higher operating empty weight (OEW) than their kerosene-fueled counterparts. Companies like Airbus are already working on designs that could accommodate this, but these designs only prove more viable for larger, long-range aircraft. It seems a promising technology for the long-haul market, but the question remains when adoption will commence, especially given the (rightfully) thorough certification process for aircraft.
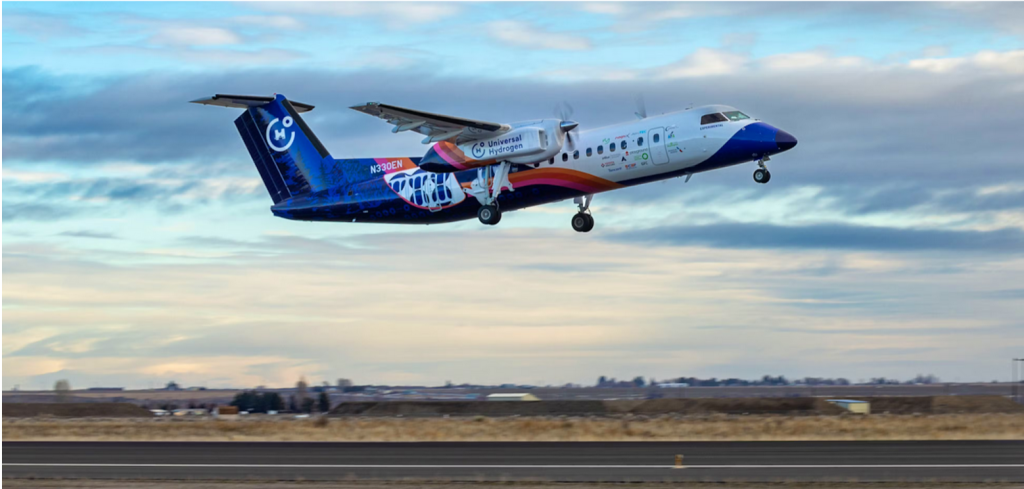
The discussion of battery-electric and hydrogen aircraft presented above leads to the conclusion that very short-haul flights (up to 500 km) are the only ones that could be decarbonized impactfully in the following five to ten years. Battery chemistry and design improvements can extend the range of battery aircraft, expanding its share of the short-haul market (500-1500 km). It is unsure whether technology can cover ranges longer than that in a reasonable timeline. Hydrogen aircraft could be viable for long-range flights, but only technological demonstrators at a subsystem level are currently in development, so a timeline for implementation is uncertain.
Already in 2024, operators have signed orders for battery-powered aircraft for very short-haul flights (up to 500 km), representing 30% of all flights – barely 4.3% of the sector emissions [7]. In contrast, short-haul flights above 500 km account for 20.5% of the total emissions, while long-haul flights account for 75.1%.
As a benchmark for 2050, Eurocontrol’s Aviation Outlook estimates an adoption rate of “revolutionary technologies”, including battery-electric, hydrogen, and hybrid aircraft, of around 8% [15]. For short-haul flights, this adoption rate would result in a reduction of 8% of emissions, since we assume battery-powered aircraft to be non-emitting. Of course, this also assumes that the battery improvements discussed above allow significant range increases beyond 500 km. For long-haul flights, assuming hydrogen is still produced through SMR, this would result in 8% of flights reducing their emissions by 75% – a total emissions reduction of 6%. Overall, total emissions reductions would hover around 6.5%, resulting in a carbon intensity of 0.248 kilograms of CO2 per kWh – far from the considerable reductions required to reach net zero.
The “Destination 2050” document states a goal of reaching net zero CO2 emissions by 2050 from all flights within and departing from the EU. How viable is this? In their 2050 outlook, Eurocontrol states that the most optimistic figures to adopt for “revolutionary technologies”, including battery-electric, hydrogen, and hybrid aircraft, by 2050 are about 8% [15]. Already in 2024, we have seen operators starting to sign orders for battery-powered aircraft for very short-haul flights (up to 500 km), which represent 30% of all flights, but barely over 4% of the emissions of the sector [7]. The reduction in emissions associated with this would thus be around 0.4% of total emissions. Mid-range aircraft account for about 45% of total emissions, and long-range aircraft account for 51. Assuming that the lower end of the mid-range (500-1500km) is covered by electric aircraft (with their assumed emissions being 0) and that both the high-mid range and the long-range aircraft are powered by hydrogen (assumed to reduce emissions to a quarter of current standards), the reductions in emissions amount to 1.84% from electric aircraft and 1.46% from hydrogen aircraft, yielding a total decrease of 3,7% of total emissions, or 0,255 kgCO2/kWh
Discussion
In this article, we studied only the effect of aviation on global warming through carbon dioxide emissions, without including non-CO2 effects, which have an important impact. Additionally, we limited our modeling of the impact on the Earth system to global warming. Rockstrom et al.’s planetary boundaries framework adds other coordinates to the discussion, such as eutrophication, land use, and material use [16]. Currently, aviation is highly carbon intensive but does not majorly impact other planetary boundaries. However, as discussed below, developments in the sector could result in outsized impacts.
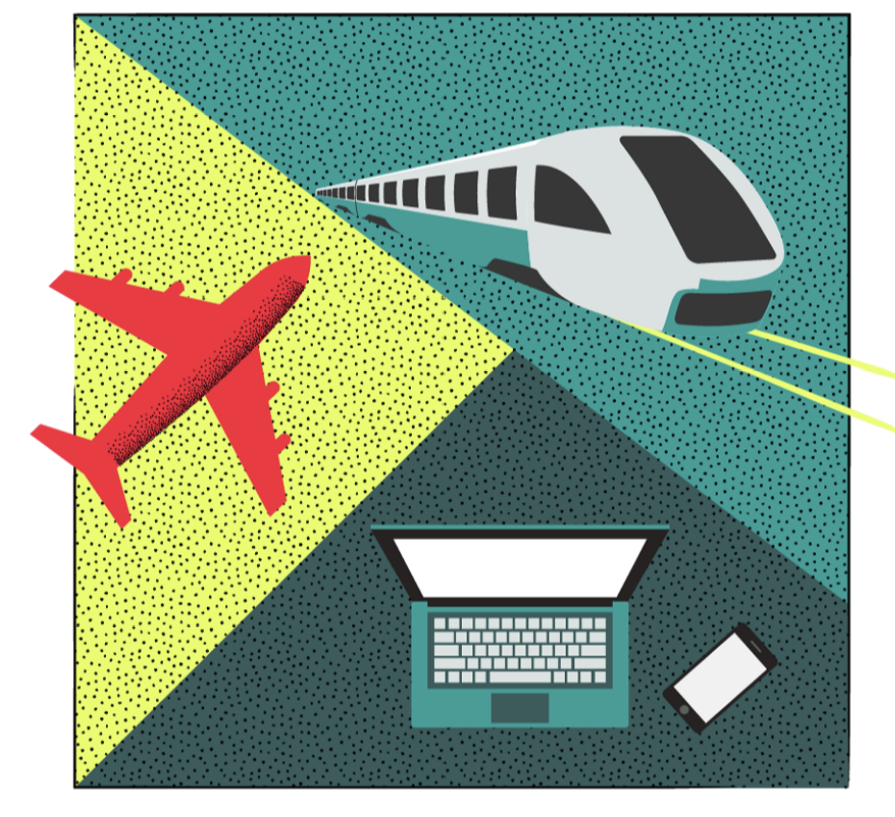
When discussing demand, we relied partially on IATA’s 2024 report. In this report, the Association proudly claims the industry’s determination to achieve net zero carbon emissions by 2050, while presenting a growth rate that would result in a tripling of overall demand, and boasting of their fight against any measures that would constrain demand. However, our back-of-the-envelope calculation shows that, short of a miracle, the only way to decrease the impact of aviation effectively is to reduce demand, or at least curtail its growth. Our results are in agreement with those found by Eurocontrol, whose Aviation Outlook for 2050 shows that only a reduction of 27% in carbon emissions would be possible without the use of SAF and offsets or carbon pricing, even in the most favorable scenario for technological evolution and revolution [15].
The IATA also estimates that 65% of carbon mitigation needed will come from sustainable aviation fuels, which, as seen above, currently represent only 0.2% of total fuel used. This means that an enormous increase in SAF is required, which means a corresponding increase in the growth of crops to produce this SAF. The future decreases in emissions depend entirely on the development of SAF infrastructure, including energy crops, which compete with agricultural crops and can induce deforestation and further emissions due to land use change and intensive fertilizer use.
The other two aspects of the trifecta of IATA solutions to close the emissions gap are represented by offsets and direct air capture. Offsets would determine the development of net environmental carbon sinks to compensate for the emissions caused by aviation and are found by Joseph Romm to be “unscalable, unjust, and unfixable” [17]. This is because current offsets are typically either not real (fraud), not additional (carbon reductions would have occurred without the revenues from the offsetting sale), or over-credited. Direct air capture is also considered necessary to complement SAF, but it is incredibly energy-inefficient, resource-intensive, and unproven at scale [18]. This does not mean that negative emissions technology should not be developed, but rather that it seems unsound to continue emitting while hinging on the possibility of a later drawdown.
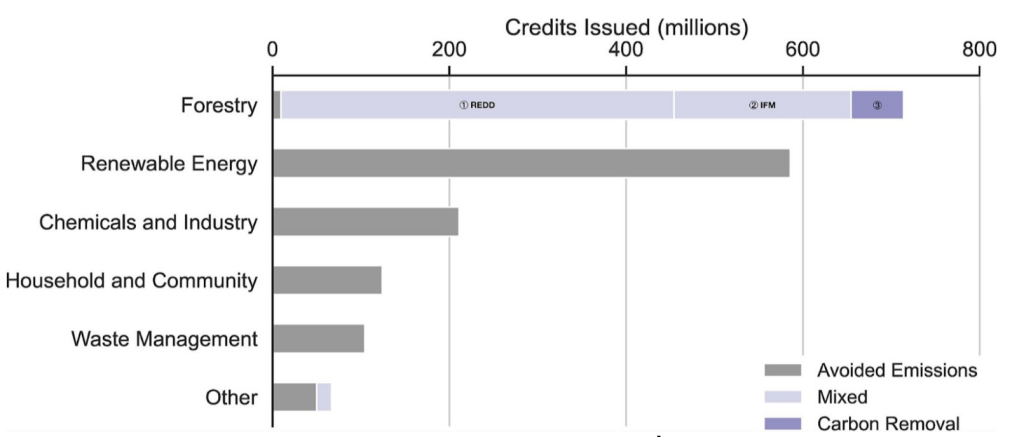
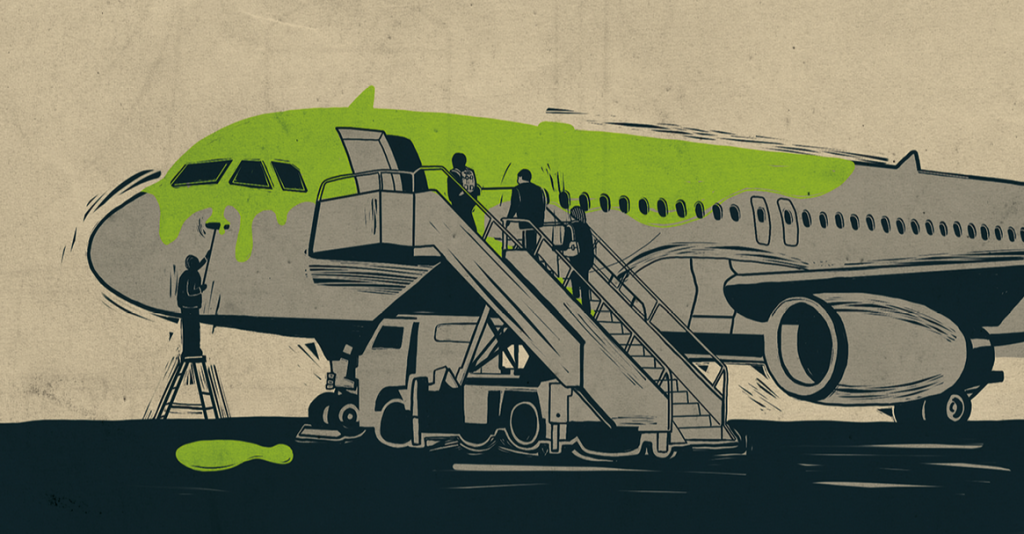
Currently, hydrogen production is overwhelmingly dominated by so-called “grey hydrogen”, produced by Steam Methane Reforming (SRM). The emissions associated with SMR are even worse than electricity production with natural gas, and the production cost of “green hydrogen” (produced by electrolysis with green electricity sources) is still high, as the technology is not mature at a large scale. There remains a great barrier to entry in the form of a high infrastructure cost. However, as oil extraction is expected to become more difficult and costly [19], hydrogen production with low-cost green electricity may become more economically viable.
Critically, our simple framework does not model cost in any way. Historically, all efficiency improvements have led to increased travel not decreased emissions because airlines are encouraged to increase profits, with the optimum point being wherever maximum revenue can be extracted. This, once again, leads to the conclusion that demand must be curtailed through policy instruments.
So how can demand be limited? Typical policy instruments such as carbon pricing, beyond being practically impossible to coordinate at a global level, naturally result in increased ticket costs, creating distributional inequalities. Seeing as 1% of the world population has caused 50% of aviation CO2 emissions [20], measures such as frequent flier levies and air miles levies [21], as well as stopping current tax exemptions, may be necessary to decrease aviation demand while raising funds for decarbonization and climate mitigation. However, these frequent fliers represent affluent and powerful parts of the world, and it remains unlikely for such a ‘self-tax’ to be dictated by the Global North.
More positively, modal shift could represent a solution for short-range flights. High-speed rail can represent a clean and comparable solution (for example, Amsterdam to Paris in a Eurostar vs. a KLM Cityhopper), but requires significant infrastructure investments. The COVID-19 pandemic brought online work to unprecedented prominence, and there is no reason why organizations cannot change their travel policies for work-related reasons, such as meetings and conferences. Increased visibility of the climate impact and decarbonization struggles of aviation may lead to further behavior change, which, combined with responsible, coordinated policy instruments, could lead to significant decreases in air travel and subsequent reductions in emissions.

In short, our excursion into the realm of decarbonization should make one thing clear: decarbonizing aviation, though possible, represents a wicked problem with contradicting incentives for the many stakeholders involved. Airlines and original equipment manufacturers are likely to welcome growth in demand, as their profitability rises with every flight and aircraft sold respectively. However, unlike other sectors, such as energy and transport, it seems that short of vigorous global public intervention, aviation is barreling toward a greater and greater impact on our climate.
Conclusion
As members of the aerospace engineering community, we believe we should not be complacent with the idea that, eventually, aviation will decarbonize. A significant system change of this magnitude requires all hands on deck and, though our main contribution will be in the realm of technological development, even our simple framework shows that it is insufficient. Only if we add our strength to the chorus of voices critical of our current path, and speak up about the necessity of systemic change, can we truly make aviation decarbonize.
References
[1] Annual Review 2024. (2024) International Air Transport Association. iata.org.
[2] Lee, D.S., Fahey, D.W., Skowron, A., Burkhardt, U., Chen, Q., Doherty, S.J. et al. (2020). The Contribution of Global Aviation to Anthropogenic Climate Forcing for 2000 to 2018. Atmospheric Environment 244 (September), 117834. doi.org/10.1016/j.atmosenv.2020.117834.
[3] Bigo, A. (2020). Transport Facing the Challenge of Energy Transition Explorations: Between the Past and the Future, Technology and Sufficiency, Acceleration and Slowdown. PhD Thesis, Institut Polytechnique de Paris.
[4] Börjeson, L., Höjer, M., Dreborg, K., Ekvall, T., and Finnveden, G., (2006). Scenario Types and Techniques: Towards a User’s Guide. Futures 38 (7), 723–39. doi.org/10.1016/j.futures.2005.12.002.
[5] Bergero, C., Gosnell, G., Gielen, D. et al. (2023). Pathways to net-zero emissions from aviation. Nat Sustain 6, 404–414. doi.org/10.1038/s41893-022-01046-9
[6] Air Travel Forecast to 2030: The Recovery and the Carbon Challenge. (2024). Bain. bain.com.
[7] EUROCONTROL Data Snapshot. (2021). Eurocontrol. eurocontrol.int.
[8] Calculation Principles – Flight Emissions Calculator. (n.d.). myclimate.org.
[9] Ehsani, S., Sergeeva, E., Murdy, W., and Fox., B. (2024). Predicting the Skies: A Novel Model for Flight-Level Passenger Traffic Forecasting. arXiv.Org. January 7, 2024. arxiv.org/abs/2401.03397.
[10] Zheng, X. S. & Rutherford, D. (2020) Fuel Burn of New Commercial Jet Aircraft: 1960 to 2019. ICCT theicct.org/sites/default/files/publications/Aircraft-fuel-burn-trends-sept2020.pdf.
[11] Resolution A40-18: Consolidated Statement of Continuing ICAO Policies and Practices Related to Environmental Protection–Climate Change. (ICAO, 2019).
[12] Aba, M. M., Sauer, I. L., & Amado, N. B. (2024). Comparative review of hydrogen and electricity as energy carriers for the energy transition. International Journal of Hydrogen Energy, 57, 660–678. doi.org/10.1016/j.ijhydene.2024.01.034.
[13] Hydrogen fact sheet. (n.d.). H2Data h2data.de.
[14] Warwick, G. (2020, October 28). The Week in Technology. Aviation Week Network. aviationweek.com.
[15] Aviation Outlook 2050: air traffic forecast shows aviation pathway to net zero CO₂ emissions. (2022, June 17). Eurocontrol. eurocontrol.int.
[16] Rockström, J., Steffen, W., Noone, K. et al. (2009). A safe operating space for humanity. Nature 461, 472–475. doi.org/10.1038/461472a.
[17] Hanley, S. (2023). “Carbon Offsets Are Unscalable, Unjust, & Unfixable — Joe Romm.” CleanTechnica. cleantechnica.com.
[18] Chatterjee, S., Huang, KW. (2020). Unrealistic energy and materials requirement for direct air capture in deep mitigation pathways. Nat Commun 11, 3287. doi.org/10.1038/s41467-020-17203-7.
[19] Misra, S. (2023, March 20). Plummeting “Energy Return on investment” of oil and the impact on global energy landscape. JPT. jpt.spe.org
[20] Gössling, S., Humpe, A.. (2020). The Global Scale, Distribution and Growth of Aviation: Implications for Climate Change. Global Environmental Change 65 (November): 102194. doi.org/10.1016/j.gloenvcha.2020.102194.
[21] Committee on Climate Change. (2019). Behaviour Change, Public Engagement and Net Zero (Imperial College London) Climate Change Committee. theccc.org.uk.[22] EUROCONTROL Aviation Outlook 2050. (2022, April 13). Eurocontrol. https://www.eurocontrol.int